WHITE PAPER
Understanding Laser Sources for 2- and 3-Photon Excitation
A whitepaper by Marco Arrigoni (Director of Strategic Marketing) and Erin Dlugosz (Product Line Manager, Monaco 1300) at Coherent
The benefits of 2- and 3- photon excitation
Two-photon excitation is a well-established tool for three-dimensional imaging that is now widely used in life sciences, particularly in the field of neuroscience. An ultrafast (usually femtosecond) laser is focused to a small beam waist. The high peak intensity at this location, and only this location, is able to drive two-photon excitation of fluorescent dyes, proteins, and endogenous materials. By scanning this laser spot in xyz, a two-dimensional image slice or three-dimensional image cube can be built up with high signal-to-noise ratio and minimal thermal impact on live tissue.
In the pursuit of deeper neuron mapping within the cortex, 3-photon excitation has gained traction in the neuroscience community. The scattering and absorption characteristics of most tissues offer several windows of high penetration depth at the wavelengths of interest. The higher-order nonlinear excitation also yields a more confined, higher intensity focus in the tissue sample resulting in clean images with virtually no out-of-focus fluorescence background. Together, these inherent characteristics of 3-photon excitation double the functional in-vivo imaging depth compared to 2-photon techniques.
2-photon and 3-photon excitation each have their own unique laser requirements in terms of pulse energy, wavelength, repetition rate, and pulse width. As such, the fundamental 2- photon and 3-photon experimental techniques cannot be addressed by the same light source. The goal of this paper is to explore these specific laser requirements in depth, highlighting how they enable each type of excitation and presenting corresponding laser developments from Coherent that streamline both 2-photon and 3-photon applications.
Laser requirements for 2-photon microscopy
Two-photon excitation of biological samples requires short pulses, high repetition rates for fast raster imaging, average powers compatible with sample viability, and wavelengths that match the excitation spectra of the many different available probes. The pulse duration (pulse width) requirement originates from the need for very high peak power due to the low probability of two-photon excitation. Pulse durations commonly used are between 75 and 250 fs, where shorter pulses result in more efficient excitation but with a higher risk of non-linear sample or even ablative damage.
Such short pulses can be produced only by operating a laser in the mode-locked regime. Most mode-locked lasers operate at fixed repetition rates of 50-100 MHz, which is a very good match for many imaging studies. For example, consider a typical image size of 512 x 512 pixels and a frame rate of a few Hz. The need to have multiple excitation pulses in each pixel to decrease noise and the overhead for raster scanning requires repetition rates > 10 MHz. Faster resonantly scanned experiments need 10s of MHz.
The most commonly used indicators are excited in the single-photon regime with wavelengths between 400 nm and 550 nm (violet to green light). In the two-photon regime this corresponds roughly to wavelengths between 750 nm and 1100 nm (near infrared), that happen to be a perfect match for tunable lasers based on a Titanium-sapphire active medium.
Table 1: Summary of optimum laser parameters for multi-photon excitation.
Laser Parameter | 2P Imaging Requirements | 3P Imaging Requirements |
Wavelength Range (nm) | 700 - 110 |
1300 - 1700
|
Average Power (W) | 1 - 2 | 1 - 4 |
Pulse Width (fs) | 75 - 150 | 40 - 60 |
Pulse Energy | 10 - 50 nJ | 1 - 2 µJ |
Repetition Rate (MHz) | 50 - 100 | 1 - 4 |
Finally, it has been shown in many different studies that most samples tolerate average laser powers of 100-200 mW at these near infrared wavelengths which is sufficient power to deliver bright images with a large variety of today’s imaging and functional fluorescent probes. With some microscopes having a throughput as low as 10%, this translates into a requirement for 1-2 Watts of laser power.
As summarized in Table 1, these requirements are well met by single box tunable and automated Titanium Sapphire (Ti:S) lasers such as the Chameleon series from Coherent. Altogether, it is estimated that there are over 5,000 of this type of Ti:S lasers used for two-photon microscopy in laboratories across the globe.
Laser source requirements for 3-photon microscopy
Compared to 2-photon microscopy, 3-photon microscopy is still in its relative infancy. But because it offers several advantages for deeper imaging (e.g., in neuroscience), its use is growing quite rapidly.
The laser requirements are determined by the much lower probability of a 3-photon event which therefore requires a considerably higher peak power than for 2-photon excitation: ~ 100-200 times higher. Higher peak power can be achieved by using a laser with shorter pulse widths and/or higher pulse energies. However, dispersion management – avoiding distorted and stretched pulses – becomes a problem when transmitting the shortest available mode-locked (e.g., 10 fs) pulses through microscope systems. Consequently, the use of 40-60 fs pulses for 3-photon excitation provides a good balance between high peak power and reasonably simple pulse management.
This pulse duration is ~ 3 times shorter than the typical pulse duration of a 2-photon laser and it means that we still need a 40-50 X increase in pulse energy compared to these lasers. Whereas 2-photon microscopy lasers have output pulse energies in the 10-40 nJ range, 3-photon microscopy with similar optical losses will require 0.5-2 mJ at the laser output.
One way to reach this pulse energy is to amplify the output of a Ti:S laser using a technique called chirped pulse amplification or CPA. CPA was invented by Gerard Mourou and Donna Strickland in the 1990s; it is so important and ubiquitously used that it earned them the 2018 Nobel prize for Physics.
Ytterbium is the key to power scaling
What about the repetition rate and average power? Here a major consideration is sample viability. It is well-established from numerous 2-photon studies that the average power on the sample should be kept below 200 mW to maintain sample viability. In the previous section, we indicated that the energy per pulse required for 3-photon imaging should be 40-50 times higher than in case of 2-photon excitation. This means that the repetition rate will have to be accordingly lower – say 1-2 MHz. For faster imaging, the repetition rate could be increased to 4-5 MHz, but only if the energy per pulse on the sample is proportionally decreased.
Unfortunately, the physical properties of Ti:S crystals make building such a laser impractical.On the other hand, a laser system based on an ytterbium-doped fiber can bypass these problems. This is because the use of a Yb-doped active fiber distributes the amplification of the pulses over a longer distance (the fiber can be coiled to result in a compact configuration) providing sufficient gain also at those high repetition rates that are unachievable with a Ti:S amplifier.
Fig 1 schematically illustrates the typical architecture of this type of Yb fiber amplifier. As with Ti:S lasers amplifiers, the first stage (seed laser+ pre-amplifier) produces a stream of mode-locked pulses at a few tens of MHz. An acousto-optic modulator (AOM) gates this pulse train and drops the repetition rate by an integer number N. With reference to the figure, if N = 50, a 1 MHz pulse train will be amplified by CPA in the power amplification stage.
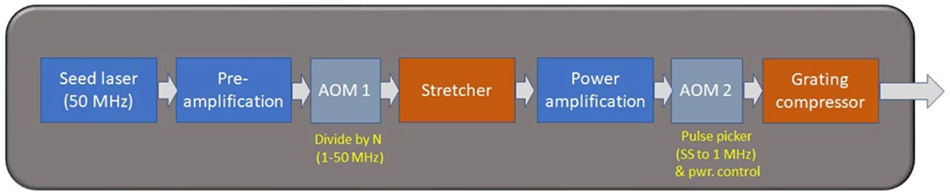
Figure 1: Block diagram of the main elements of a high-power Ytterbium fiber laser.
This architecture is extremely flexible and power-scalable; it can readily produce average powers up to 60 watts and beyond, supported at any repetition rate from 1 MHz to 50 MHz. The energy per pulse is limited by the onset of damage to the optical fiber, and typically kept below 100 mJ per pulse.
Yb lasers based on CPA operate at a fixed wavelength of 1030-1070 nm and their gain bandwidth can support pulses of ~250 fs. Neither wavelength or pulse duration are directly compatible with 3-photon microscopy and therefore additional pulse processing is required as described in the next section. As a side note, these Yb lasers have become extremely popular for 2-photon optogenetics with so-called red-shifted opsins, ie., excitable at the Yb laser wavelength where their high output power is “spread” over many neurons using spatial light modulators.
Wavelength requirements for 3-photon excitation
For 3-photon microscopy, the wavelength required to excite probes based on green fluorescent protein is ~1300 nm; for red-shifted probes it is ~1700 nm. Of these two wavelength regimes, 1300 nm is proving to be the more beneficial 3-photon imaging “window”, a point which will be expounded later in this paper. In addition, efficient 3-photon excitation also requires pulse widths of 40-60 fs. So how do we get this performance using Yb lasers?
The most popular method of transforming both the wavelength and pulse width to meet the requirements for 3-photon microscopy is to use a wavelength-tunable optical parametric amplifier (OPA). This device converts the pump wavelength (from the Yb laser) into two longer wavelengths, where the sum of their photon energy equals the photon energy of the pump light. This conservation of energy can be expressed as:
1/λp=1/λs+1/λi
where λ denotes the wavelength and p, s and i identify the “pump” wavelength and the two wavelengths produced by the OPA that are conventionally called “signal” and “idler” wavelengths. For historical reasons, the idler is always the shorter of the two wavelengths.
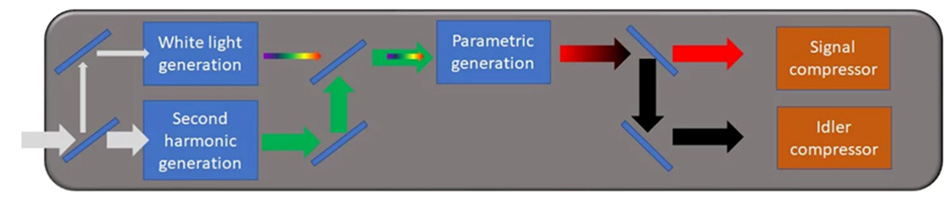
Figure 2: Block diagram of the main elements of an OPA.
Figure 2 illustrates schematically how the OPA generates the requisite wavelengths for 3-photon microscopy. A small part of the Yb laser output is used to produce a broadband “white light continuum” in a thin plate. The white light then gets amplified in a non-linear crystal suitable for parametric amplification at a specific wavelength selected by the user. The rest of the Yb laser output is frequency-doubled to create green light at 515-520 nm, which is the “pump” wavelength of the OPA. With this arrangement, the OPA can produce - for example - an idler wavelength of 1300 nm, which matches 3-photon excitation of popular “green” probes. Energy conservation requires that the signal wavelength be 860 nm.
Given the white light nature of the continuum, how does the OPA user select the two output wavelengths? This is achieved by phase-matching, i.e., adjusting the angle between the optical axis of the crystal and the pump beam. By tilting the crystal, it is possible to select which pair of wavelengths from the white light will be amplified. Tunable OPAs have a manual or motorized and computer-controlled stage to simplify selection of the signal wavelength of choice.
Matching the pulse width requirement
Just as important for 3-photon microscopy, the specific design of the OPA enables the production of pulses that are much shorter than the pump pulse. Specifically, by using a “non-collinear” design, where the pump beam and the signal beam propagate at different angles in the OPA crystal, pulses as short as 20-25 fs can be produced i. This non-collinear approach leaves a ~300 nm gap in the tuning range, typically between 940 and 1250 nm. Fortunately, this region is not relevant for 3-photon excitation.
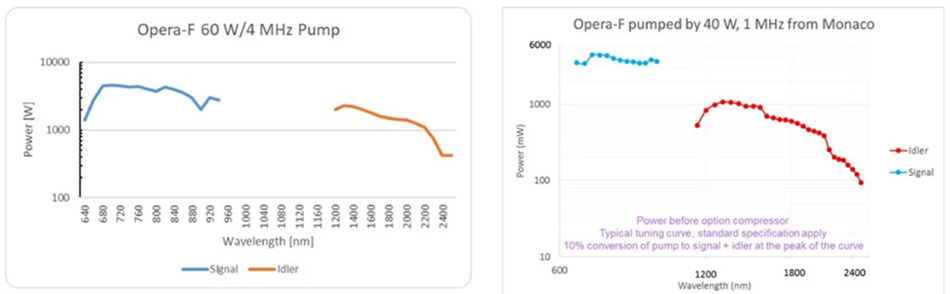
Figure 3: Tuning curves of Opera-F for 40 and 60 watt pumping with the Coherent Monaco.
Because of the non-linear processes involved (frequency doubling of the Yb laser and parametric generation), the overall system efficiency is relatively low. That’s where the power scaling of Yb lasers pays off. A powerful Yb laser like the Coherent Monaco delivers a few tens of µJ per pulse. This yields a few µJ per pulse from an OPA at 1300 or 1700 nm, which matches the optical “windows” for 3-photon excitation. Fig. 3 shows a typical tuning curve for an OPA (Coherent Opera-F) pumped with 60 Watts at 1 and 4 MHz, a set-up used by many research groups.
Simultaneous multi-probe imaging
In the previous sections we made reference to 1300 nm as the key wavelength to excite in a 3-photon modality important probes like green fluorescent proteins and the GCaMP family of genetically encoded Calcium indicators, where 3 photons carry the same energy as 2 photons at 920 nm. Similarly, so-called “red-shifted” probes that are excited at ~1050 nm using 2-photon excitation, can be excited in 3-photon modality at 1600- 1700 nm. These probes include the m-Fruit morphology probes and the RCaMP Calcium probes – See also fig.4
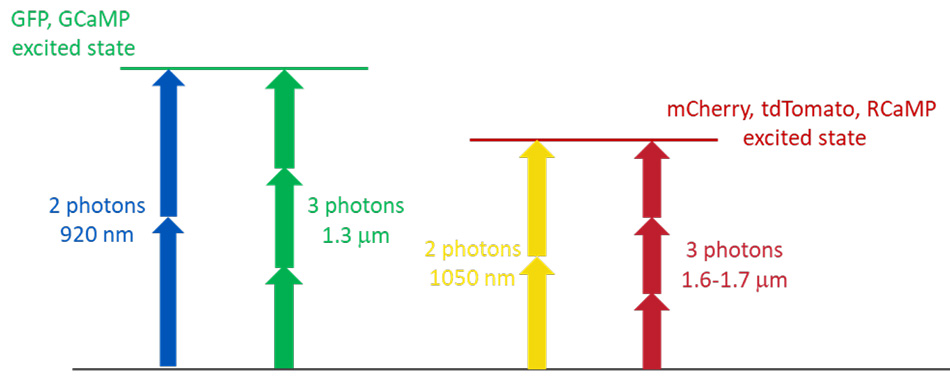
Figure 4: 2- and 3-photon excitation of green and red-shifted probes.
While the vast majority of 3-photon work has been conducted at 1300 nm, the use of red-shifted probes notionally excitable at the longer wavelength of 1600- 1700 nm is still of interest in the microscopy community. This seems to indicate that an ideal 3-photon laser should be able to produce both wavelengths, maybe even at the same time, in case of two-color excitation imaging. The longer wavelength has the potential for even deeper imaging because of the lower tissue scattering, although water absorption is higher at 1700 nm than at 1300 nm, meaning there is a higher probability of sample thermal damage.
However, several published studies have shown that 1300 nm can be used to excite both green and red fluorescent probes, or at least, a number of them. The fluorescence emission from the two probes is then easily separated using wavelength selective filters. For example, in 2021, Chris Xu published a landmark article on single wavelength 3P excitation of multicolor fluorophores (Multicolor three-photon fluorescence imaging with single-wavelength excitation deep in mouse brain | Science Advances).
More recently, Timo van Kerkoerle and his Ph.D. student Marie Guillemant accomplished simultaneous excitation of mouse interneurons labeled with both dextran and tdTomato (a red-shifted probe) in mouse prefrontal cortex via 3P excitation with a Coherent Monaco and Opera-F tuned to 1300 nm (Figure 5). The z-stack image in Figure 4 shows significant signal from the red-shifted probes down to a depth of ~1 mm.
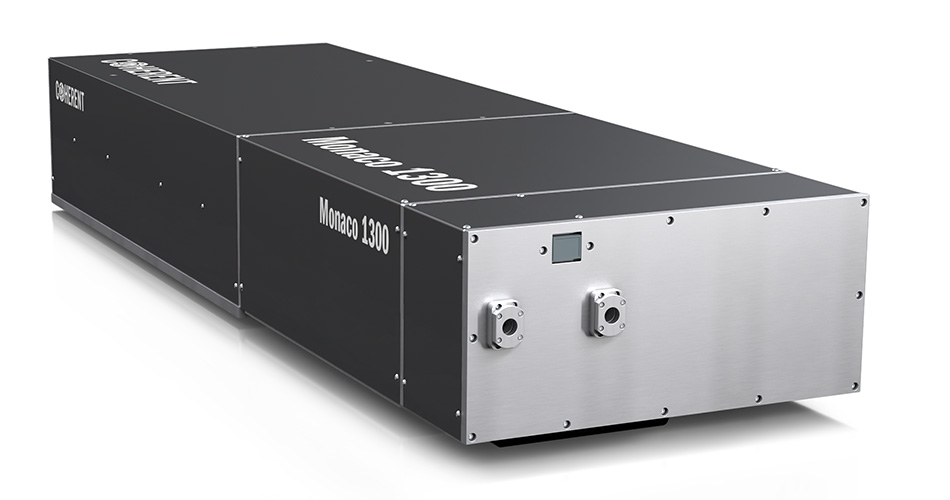
A Coherent Monaco 1300 laser head containing pump laser, 1300 module with Total Power Control option, and Compact Pulse Compressor option all fully integrated in one box.
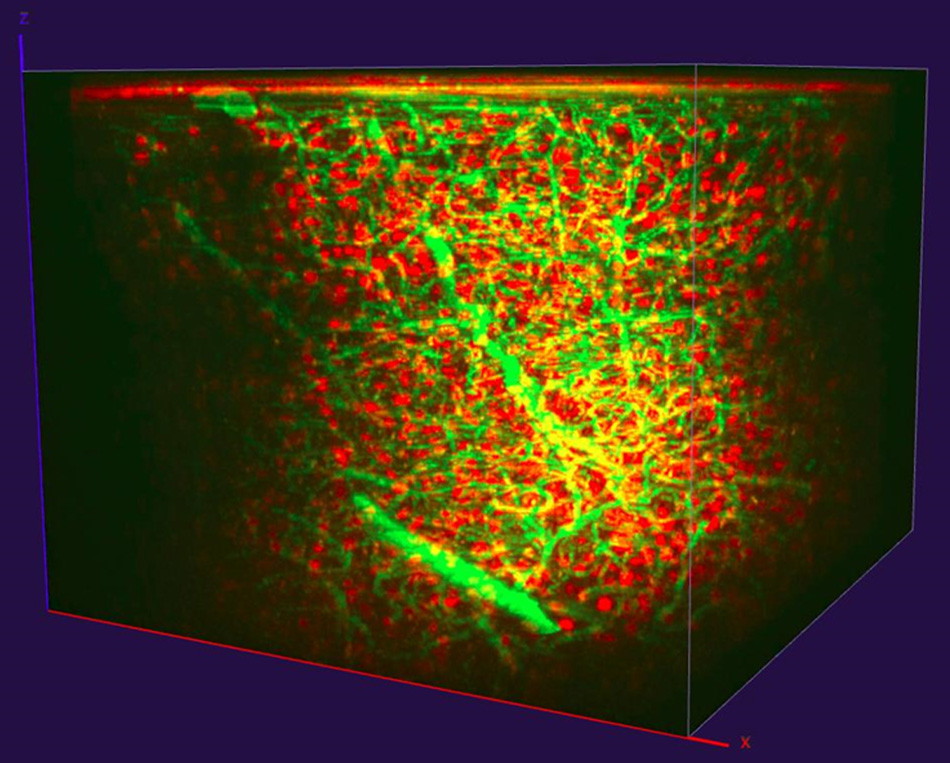
Figure 5: 3P imaging of dextran (green) and tdTomato (red) labelled interneurons in mouse prefrontal cortex, reaching ~1 mm depth. Data courtesy of Timo van Kerkoerle, Ph.D. and Marie Guillemant, Neurospin, CEA Saclay.
This approach not only eliminates the need for two OPAs, it also eliminates the need for an OPA with wavelength tuning. To enable easier implementation of this multi-probe approach, Coherent has introduced a one-box, fixed wavelength source of 1300 nm femtosecond pulses, called the Coherent Monaco 1300. This compact source is a fully integrated, hands-free laser delivering up to 2.5 W of output power, with a choice of 1, 2, or 4 MHz repetition rates, and a pulse width of <50 fs. All these parameters are an ideal match for deep 3-photon imaging, so that the Coherent Monaco 1300 is the first single-box, turn-key light source specifically addressing 3-photon microscopy.
Recognizing the emergence and increased popularity of 3-photon imaging, the design of the Coherent Monaco 1300 incorporates user-benefits that are part of the most advanced lasers for 2-photon microscopy, like the Coherent Chameleon Discovery and Axon. In, fact, the Coherent Monaco 1300 features in a single-box the Total Power Control (TPC) option providing on-the-fly power attenuation/gating, and the Compact Pulse Compressor (CPC) option providing dispersion precompensation for optimum pulse width at the sample with any type of microscope set-up.
Summary
In summary, multiphoton microscopy is an incredibly dynamic field driven by neuroscience and intravital imaging. The need for deeper imaging, especially in neuroscience, has already resulted in two generations of specifically designed lasers, with the first one represented by the Coherent Monaco/Opera-F configuration and the second, most recent one by the unique Coherent Monaco 1300 integrated system. While it’s too early to say if only one or two wavelengths will be mainstays in 3-photon imaging, Coherent offers a flexible solution addressing both optical windows and an integrated solution for 1300 nm that simplifies the overall microscope set-up and enables further system integration along the same lines of the single-wavelength Coherent Axon laser sources for 2-photon imaging.